It has been exactly a decade since Emmanuelle Charpentier and Jennifer Doudna demonstrated that the CRISPR system from the bacterium Streptococcus pyogenes can be harnessed to edit DNA1. Since that moment, CRISPR has quickly become a widespread genetic tool to manipulate the genomes of countless organisms. Importantly, it has revolutionized R&D across all life sciences areas—including agriculture, medicine, bioenergy, biochemicals, and biomaterials—and some exciting applications are starting to be commercialized. It was, therefore, no surprise that Charpentier and Doudna later received the Nobel Prize in Chemistry in 2020.
While “CRISPR” is now a commonly recognized word that has become synonymous with gene modification, there is much confusion around what CRISPR currently allows us to do versus what it could enable in the future. This Fieldnote aims to outline the major challenges of the first generation of CRISPR (“CRISPR 1.0”) before laying out applications that are most promising for agriculture.
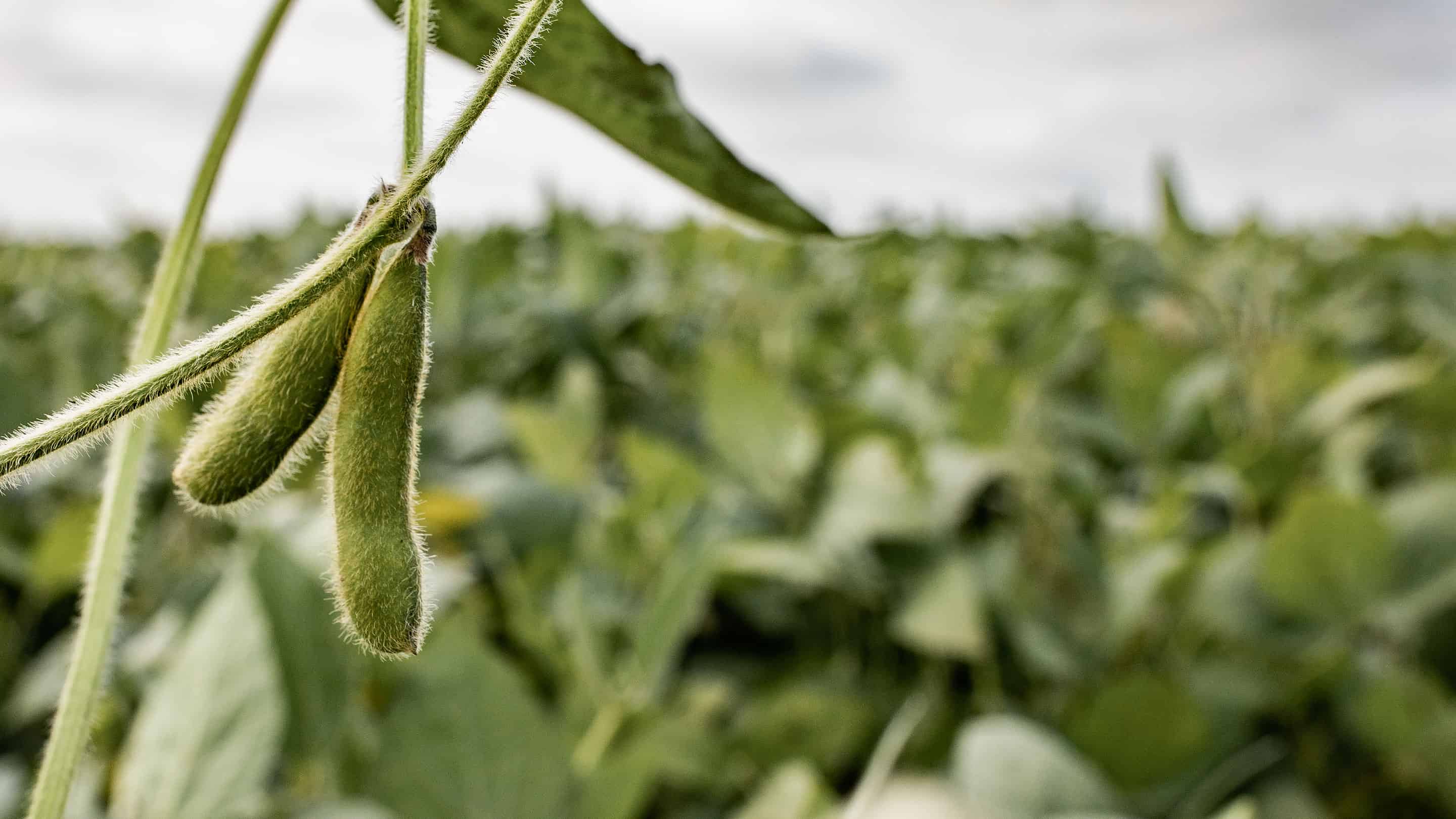
CRISPR 1.0
At its core, CRISPR—or Clustered Regularly Interspaced Short Palindromic Repeats—is a defense mechanism that prokaryotic microbes (bacteria and archaea) have evolved to combat viral infections. When foreign genetic material is detected, microbes integrate fragments of it into their own genome to create a record of the invasion. This acquired knowledge is then used to design a CRISPR weapon against the virus. Specifically, the microbes produce RNA that is complementary to the invasive genetic material and that guides a molecular scissor, called Cas, to the invasive material. Once in contact with the foreign DNA or RNA, Cas cuts it to suppress the virus. The beauty of this system is its incredible versatility: it can learn to specifically recognize innumerable genetic sequences.
Today when we talk about CRISPR, we are talking about the set of molecular tools that were bioengineered and inspired by this defense mechanism. These tools allow the precise recognition of a short stretch of DNA despite being “buried” within a massively larger genome. Similar to the original microbial system, this is achieved by providing the system with an artificial “guide RNA,” or gRNA, that is complementary to the target DNA. This is the most revolutionary aspect of the system: it can be easily provided with the “zip code” (the gRNA) to target a specific piece of DNA in the genome. Once the system reaches its destination (i.e., the target DNA region), the molecular scissor, Cas, makes a cut. As the organism tries to repair this DNA cut, it will often make a mistake: it will introduce point mutations (nucleotide changes) or “indels” (small DNA insertions or small DNA deletions). When targeting a gene, this mistake can be enough to dramatically alter the expression of a target gene or disrupt it altogether (See Figure; I also recommend watching this short educational video by MIT).
This ability to easily introduce mutations at precise DNA regions is by itself worthy of a Nobel prize. Historically, mutating a specific gene was a laborious and time-consuming process. In plants, it entailed using mutagens to randomly introduce mutations across the genomes of hundreds of thousands of plants with the hope that, by chance, one individual would be mutated at the desired genomic region. Alternatively, a gene variant identified in a closely related plant species could be introduced through classical breeding methods but with the significant limitation of taking years. Instead, introducing mutations via CRISPR is a significantly faster and more straightforward process. Today, the CRISPR-based introduction of mutations at targeted locations is a well-established technique in plants and it has been successfully used to design strains with new traits, including biotic and abiotic stress tolerance, herbicide resistance, higher yields, and biofortification2. On the commercial front, last year, the Japanese company, Sanatech Seed, became the first in the world to commercialize a CRISPR-edited crop. Specifically, they developed “Sicilian Rouge tomatoes,” in which genes were disabled to accumulate high levels of γ-aminobutyric acid GABA, a health-promoting compound3.
Despite these breakthroughs, CRISPR is far from having reached its full potential. First, each CRISPR system has constraints that are unique to the species from which it was borrowed. For example, the most commonly used CRISPR system is the one from Streptococcus pyogenes, which has restrictions around the types of DNA sequences it can target and the major drawback of having off-target activity. Second, as previously mentioned, the original CRISPR system is primarily limited to introducing random mutations in a target DNA region. However, the holy grail of gene editing is to be able to write in or rewrite genomic regions with any DNA sequence. This would include rewriting part of a gene to alter its function or adding an entirely new gene. While a DNA piece can be introduced with the goal that the DNA repair machinery uses it as a template to be copied into the CRISPR-induced cut, this process has poor success rates.
Today, a series of CRISPR innovations are emerging from academia that not only address these limitations but also offer exciting new tools based on bioengineered CRISPR variants. Although it may take a few more years before we experience the full impact of the new generation of CRISPR, it will be profound and far-reaching.
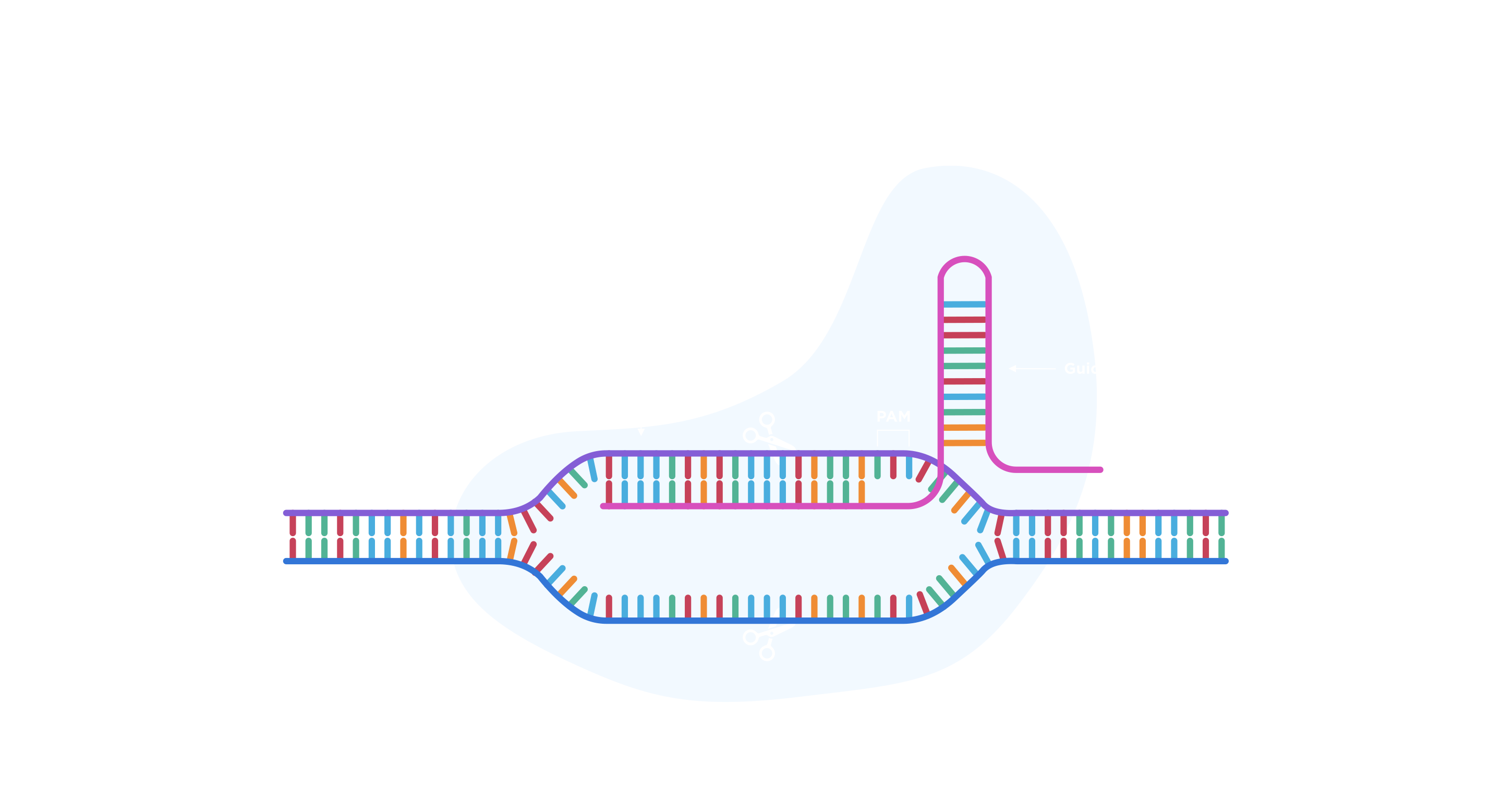
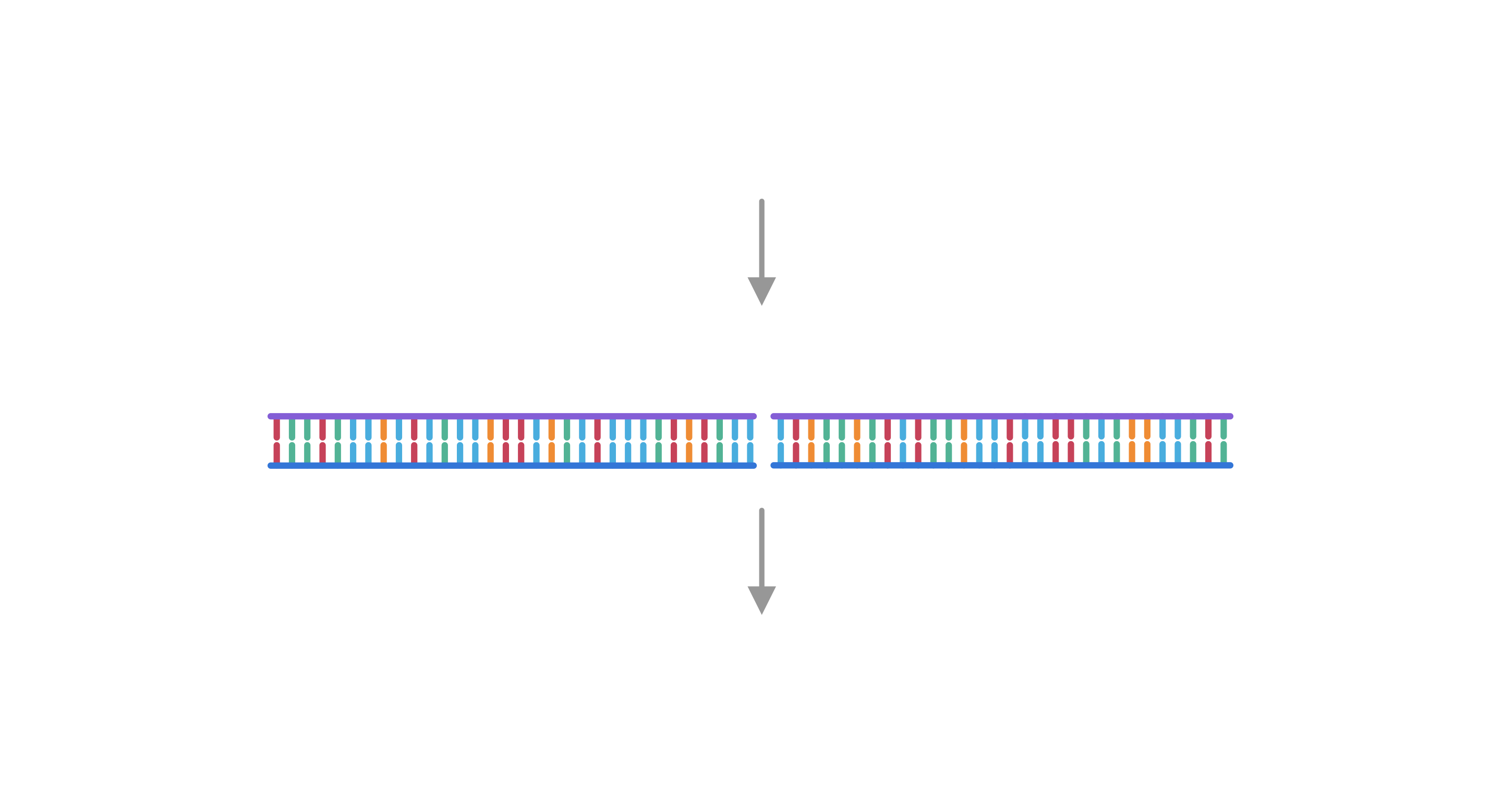

Expanding the CRISPR toolkit
As previously mentioned, the traditional CRISPR system has intrinsic issues that limit its use. Prime among them is the set of rules that the molecular scissor, Cas, adheres to. While the “zip code” it will go to can be dictated, Cas will only recognize these sequences if they are adjacent to a predefined DNA sequence, known as Protospacer Adjacent Motif or PAM (see Figure). This limits the genome’s coverage to DNA regions with a PAM sequence near them: if a gene does not have a PAM sequence in it or around it, the CRISPR system would not be able to target it.
For example, the most commonly used Cas—Cas9 from Streptococcus pyogenes—will only recognize DNA regions that are directly adjacent to an “NGG” sequence, where N can be any of the four DNA nucleotides: A, C, T or G. Therefore, while the system could recognize DNA sequences that neighbor AGG, CGG, GGG, or TGG, it will not recognize it if it is next to any other set of three nucleotides (e.g., AGA).
Paradoxically, while the CRISPR system may be too picky with regions lacking PAMs, it may still make mistakes and cut non-target areas of the genome. These off-target cuts can lead to mutations that may be particularly problematic when they occur in other genes, which could have the effect of inadvertently suppressing other traits. Off-target mutations are also problematic from a public perception and regulatory perspective. While the USDA does not consider off-target modifications a problem since, in theory, similar mutations could occur naturally4, it has complicated the debate around CRISPR-edited crops in the European Union5.
To overcome these problems, two strategies are being pursued: bioengineering Cas9 and mining microbes to identify additional Cas proteins. On the engineering front, Cas9 has been successfully engineered to either reduce its off-target effects6 or to be less limited by its PAM requirement7. Moving forward, combining these two improvements should lead to a superior Cas9 that could be readily used in established protocols. On the mining front, the goal is to take advantage of the wide diversity of CRISPR systems that have evolved in different bacteria, archaea, and bacteriophages. In fact, 33 subtypes of CRISPR systems exist, each belonging to one of 6 types and 2 classes8. They exhibit essential differences in their target recognition and mode of action, with some even targeting RNA instead of DNA. For example, a CRISPR system from Alicyclobacillus acidoterrestrisrecognizes sequences next to an “NTT” PAM, while another from bacteriophages recognizes sequences next to a “TBN” PAM where B is any nucleotide other than A. Incidentally, the latter system has the added advantage of having a significantly smaller Cas protein which facilitates its delivery9. Altogether, when optimized for expression and use in different crops, these innovations allow a considerably higher percentage of their genomes to be edited.
Killing Cas to Write In and Rewrite DNA
What makes the CRISPR system revolutionary is the ease with which different DNA regions can be precisely targeted by designing a suitable gRNA. However, it may not always be useful to cut the target DNA. A breakthrough innovation has stemmed from the realization that the Cas protein could be “killed” with mutations to prevent it from cutting while still allowing the CRISPR system to target a precise DNA region. Specifically, a CRISPR system containing a “dead Cas” can be used as cargo to deliver a wide diversity of proteins to a specific DNA region. This means that actions other than cutting can be performed when adding certain enzymes or factors to the system. One possibility is to use gene regulators and shuttle them, via a dead Cas CRISPR system, to a gene of interest to either increase or decrease its expression. This method has already been successfully employed in numerous agronomic applications, including improving drought tolerance10, enhancing plant immunity11, and modifying flowering time12.
An even more exciting application of dead Cas CRISPR systems is the use of “Prime Editors” and “Base Editors” that can rewrite or write in DNA. Base Editors can convert a nucleotide into a different one. The advantage over traditional methods is that the user no longer has to rely on random mutations but, instead, has full control over the introduced mutation. Of the twelve possible nucleotide conversions, C to T and A to G transitions are the most well-established in plants and have already proven useful in improving crop traits13. Additional transitions are currently being developed for use in plants and will soon expand our ability to rewrite individual nucleotides. “Prime Editing” goes further than Base Editing in that it allows rewriting, inserting, or deleting short stretches of DNA. Despite being relatively short, it turns out that these modifications are sufficient to regulate many important agronomic traits. In rice, for example, 85% of the 348 genomic regions responsible for agronomic differences between various rice species—such as grain yield, grain quality, and stress tolerance—can theoretically be precisely modified using prime editors14. However, despite the game-changing potential of this technology, it is still very much in its infancy, especially in plants where efficacy has been relatively low. It will therefore take a few more years before we see its widespread adoption.
Chromosomal manipulations
Traditional breeding aims to mix genetic material from two or more species/crop varieties to generate plants with superior agronomic traits. A key event that needs to occur for traits to be permanently combined is the physical exchange between chromosomes through a process known as chromosomal crossover. In this process, pairs of matching chromosomes find each other and trade chromosomal fragments. Ideally, this exchange would yield one chromosome that contains all the favorable fragments and another with the less favorable ones so that a superior progeny containing the “perfected” chromosome would emerge. In reality, it would require incredible luck for the chromosomes to be cut and stitched exactly where intended during the exchange. Instead, genes that are close together on a chromosome are hard to separate, and, during traditional breeding, a favorable gene may “drag” its neighboring genes with it. This is a problem when these neighboring genes confer undesirable traits.
CRISPR’s ability to cut DNA at precise target locations was proposed as a strategy to break genetic linkages in plants and provide control over where chromosomal exchanges occur. The idea is that by designing a CRISPR system that cuts between neighboring regions, the likelihood of a chromosomal crossover occurring between them would significantly increase. While this is yet to be demonstrated in germ (reproductive) cells, it was shown to work in somatic (non-reproductive) cells in tomatoes15 and corn16. Therefore, while still very early in its development, these early successes indicate that this could soon become a useful breeding tool.
Another breeding use case for the CRISPR technology is the rearrangement of chromosomal segments to either allow or prevent them from exchanging genetic material. For example, a common chromosomal rearrangement is an inversion, whereby a chromosomal segment gets reversed end-to-end so that if the original order of genes on the chromosome was A, B, C, D, E, and F, it would now be A, E, D, C, B, and F. Importantly, the resulting AEDCBF chromosomal segment becomes incompatible with an ABCDEF chromosomal segment and, therefore, no exchanges can occur between them. Accordingly, if a breeder wanted to replace the E gene variant from a plant carrying an ABCDEF chromosomal segment with the E’ variant from a plant carrying an A’E’D’C’B’F’ chromosomal segment, it would not be possible. A solution to the problem would be to reverse the inversion of the A’E’D’C’B’F’ segment into an A’B’C’D’E’F’ segment. This is exactly what was recently achieved using CRISPR17. By designing gRNAs on either side of the inversion (between A’ and E’, and between B’ and F’), the CRISPR system can cut out the inverted region. When the plant attempts to repair the cuts, it may “accidentally” stitch the fragment upside down. The resulting chromosomal fragment, A’B’C’D’E’F’, can then pair with the ABCDEF one and exchange genetic material. The feasibility of such chromosomal engineering in crops was recently demonstrated in a corn study18. In this study, a particularly large inversion that spanned about one-third of chromosome 2 was re-inverted. Since this inversion exists in 3 out of 66 of inbred lines the study tested, this re-inversion strategy could enable the development of additional lines by combining traits encoded in chromosome 2.
The same strategy can be applied to achieve the opposite: create genetic incompatibility between different plant lines. This was demonstrated last month in the model organism Arabidopsis thaliana where almost nine-tenths of chromosome 2 were inverted, which had the effect of largely excluding chromosome 2 from genetic exchange with non-inverted counterparts19. This can be useful when breeders wish to “lock in” certain chromosomal regions while allowing the rest of the genome to engage in genetic transfers. Another potential application is the prevention of outcrossing. By creating sweeping inversions across a more substantial portion of the genome, a given plant line can be prevented from reproducing with other lines or varieties. In fact, given that such a plant line would be genetically isolated, it could even be considered a novel plant species altogether. This could be useful in cases where a plant line must remain genetically pure, a wild species needs to be protected from outcrossing, or GMO escape needs to be prevented.
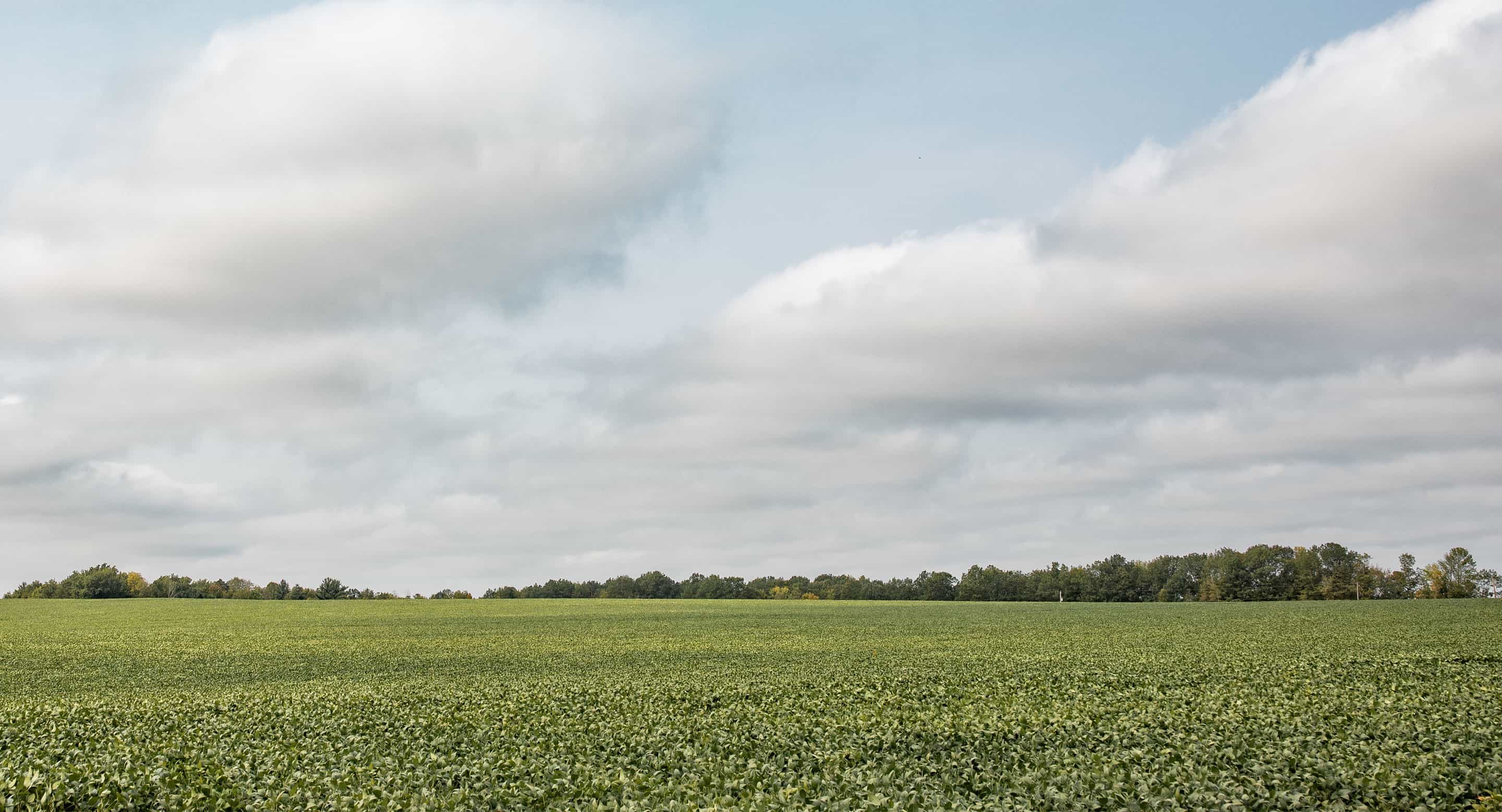
Perspective
In less than a decade, CRISPR has revolutionized plant genetics, providing researchers and crop breeders with powerful tools to mutate, manipulate, and rewrite genomes. These tools allow us to improve existing crop varieties and develop new varieties with additional traits: from biotic and abiotic stress resistances to higher yields and better resource efficiencies. Perhaps even more excitingly, CRISPR could accelerate the domestication of wild plants and underutilized “orphan crops” that play essential roles in local diets in the developing world but that are incompatible with modern infrastructure and practices. For example, in a study published last year, researchers used emerging CRISPR technologies, including base editing, to partially domesticate wild rice (Oryza alta) by modifying agronomically important traits like seed shattering, grain size, and plant height20.
Ultimately, while we can continue to expect revolutionary proof of concepts in lab settings, their translation to the field will depend on public perceptions and the implementation of safeguards to ensure responsible use. When it comes to “CRISPR 1.0”, it has the significant advantage of creating mutations that could, at least theoretically, occur in plants through natural mutagenesis. Therefore, permissive regulatory frameworks have already been established or are in advanced stages worldwide. For example, the USA and several other countries have ruled that CRISPR-edited crops are akin to conventionally bred ones, whereas the European Union is currently reassessing its 2018 decision that they should be classified as GMOs21. It remains to be seen how the new sets of CRISPR technologies will be regulated, particularly those that enable modifications significantly less likely to occur through “natural” means. Regardless of how these technologies get regulated, CRISPR advancements have provided academic research with an unprecedented toolbox to study plants. And while it may not be easily quantifiable, there is little doubt that the newly acquired knowledge will trickle into countless new agricultural applications.
1 Jinek, Martin, et al. “A programmable dual-RNA–guided DNA endonuclease in adaptive bacterial immunity.” Science 337.6096 (2012): 816-821.
2 Ricroch, Agnès, Pauline Clairand, and Wendy Harwood. “Use of CRISPR systems in plant genome editing: toward new opportunities in agriculture.” Emerging Topics in Life Sciences1.2 (2017): 169-182.
3 Waltz, Emily. “GABA-enriched tomato is first CRISPR-edited food to enter market.” Nat. Biotechnol 40.1 (2022): 9-11.
4 Hoffman, Neil E. “Revisions to USDA biotechnology regulations: The SECURE rule.” Proceedings of the National Academy of Sciences 118.22 (2021): e2004841118.
5 LAANINEN, Tarja. “New genomic techniques: European Commission study and first reactions.” (2021).
6 Lee, Jungjoon K., et al. “Directed evolution of CRISPR-Cas9 to increase its specificity.” Nature communications 9.1 (2018): 1-10.
7 Walton, Russell T., et al. “Unconstrained genome targeting with near-PAMless engineered CRISPR-Cas9 variants.” Science 368.6488 (2020): 290-296.
8 Wada, Naoki, Keishi Osakabe, and Yuriko Osakabe. “Expanding the plant genome editing toolbox with recently developed CRISPR–Cas systems.” Plant Physiology 188.4 (2022): 1825-1837.
9 Pausch, Patrick, et al. “CRISPR-CasΦ from huge phages is a hypercompact genome editor.” Science 369.6501 (2020): 333-337.
10 de Melo, Bruno Paes, et al. “Transcriptional modulation of AREB-1 by CRISPRa improves plant physiological performance under severe water deficit.” Scientific reports10.1 (2020): 1-10.
11 Khan, Zulqurnain, et al. “CRISPR/dCas9-mediated inhibition of replication of begomoviruses.” Int. J. Agric. Biol 21 (2019): 711-718.
12 Lee, Joanne E., et al. “CRISPR-based tools for targeted transcriptional and epigenetic regulation in plants.” PLoS One14.9 (2019): e0222778.
13 Hua, Kai, Peijin Han, and Jian-Kang Zhu. “Improvement of base editors and prime editors advances precision genome engineering in plants.” Plant Physiology 188.4 (2022): 1795-1810.
14 Hua, Kai, Peijin Han, and Jian-Kang Zhu. “Improvement of base editors and prime editors advances precision genome engineering in plants.” Plant Physiology 188.4 (2022): 1795-1810.
15 Filler Hayut, Shdema, Cathy Melamed Bessudo, and Avraham A. Levy. “Targeted recombination between homologous chromosomes for precise breeding in tomato.” Nature Communications 8.1 (2017): 1-9.
16 Kouranov, Andrei, et al. “Demonstration of targeted crossovers in hybrid maize using CRISPR technology.” Communications biology 5.1 (2022): 1-11.
17 Schmidt, Carla, et al. “Changing local recombination patterns in Arabidopsis by CRISPR/Cas mediated chromosome engineering.” Nature communications 11.1 (2020): 1-8.
18 Schwartz, Chris, et al. “CRISPR–Cas9-mediated 75.5-Mb inversion in maize.” Nature plants 6.12 (2020): 1427-1431.
19 Rönspies, Michelle, et al. “Massive Crossover Suppression by CRISPR-Cas-mediated Plant Chromosome Engineering.” (2022).
20 Yu, Hong, et al. “A route to de novo domestication of wild allotetraploid rice.” Cell 184.5 (2021): 1156-1170.
21 Buchholzer, Marcel, and Wolf B. Frommer. “An increasing number of countries regulate genome editing in crops.” New Phytologist (2022).